At the Dawn of the Hydrogen Economy
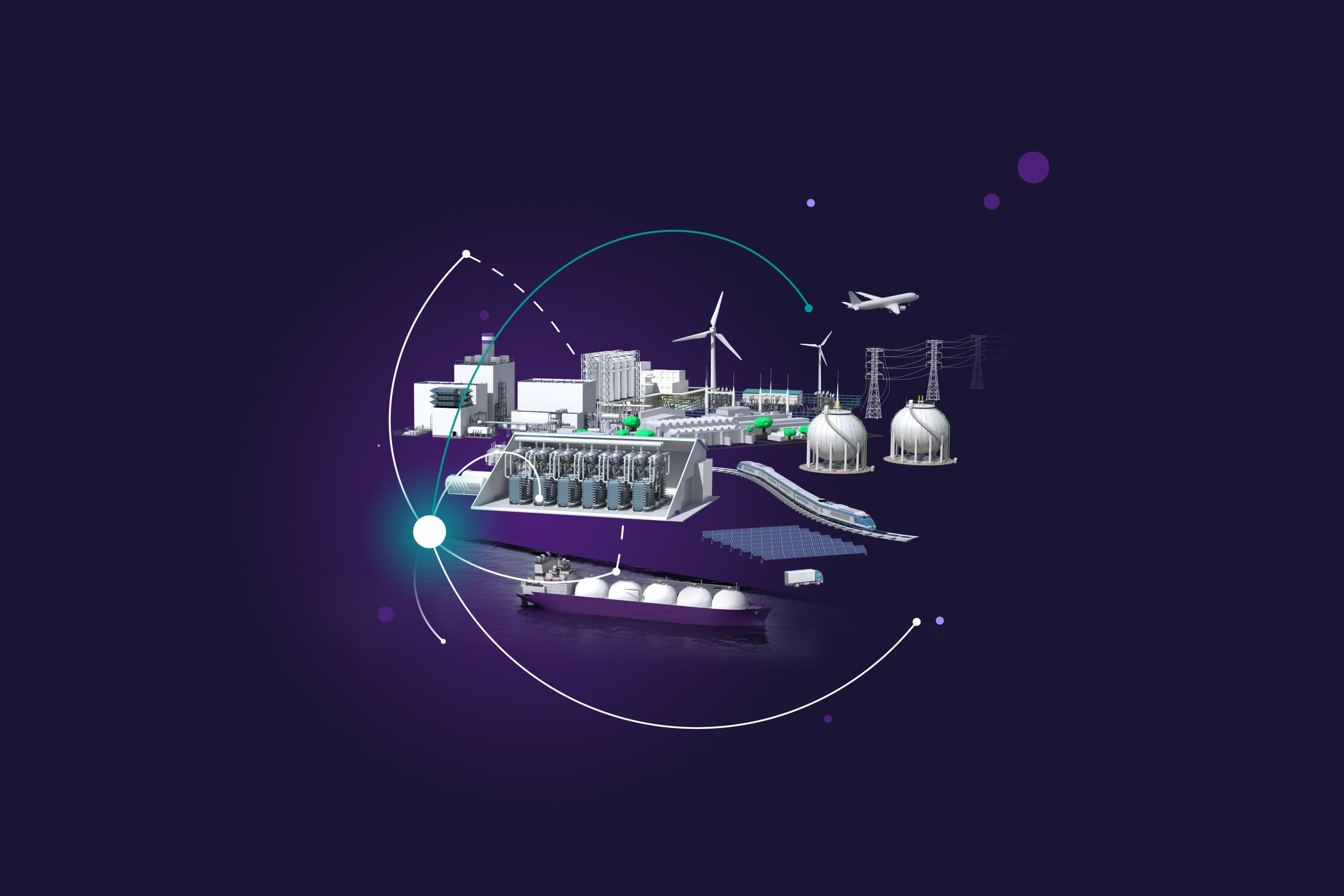
Interest in hydrogen is growing, with demand increasing rapidly. It is clear that the next significant transformation in the energy transition will be based on the hydrogen economy, transforming green electrons to green molecules via water electrolysis to create green hydrogen.
At the moment, 80 million tons of hydrogen is produced each year, and that is expected to increase by about 20 million tons by the end of the decade. Looking even further ahead, by 2050, many studies suggest production will have grown to about 600-700 million tons. Today, the majority of the hydrogen is consumed near where it is produced, most often at a chemical plant, but in the future that demand will be broader with blue hydrogen forming a bridge to what will be a green hydrogen future.

For a gas that is colorless, tasteless, and odorless, it is ironic that it is designated as grey, blue, and green; the color designated depending on the production method. For hydrogen to reach its potential as an environmentally friendly source of energy, it is vital that the supply chain is emission free.
Today's conventional method of producing hydrogen is from fossil fuels such as methane, natural gas, or coal. This type of hydrogen, dubbed grey hydrogen, comprises more than 95% of the quantity produced today, and it is an emissions-intensive process, with ~10 kilograms of CO2 emitted to produce each kilogram of hydrogen. If a CO2 capture and storage or usage process (CCUS) is added, this hydrogen albeit produced from hydrocarbons is emission free and often referred to as blue.
Siemens Energy is supporting the whole sustainable CO2 emission free hydrogen value chain that needs to be developed to achieve the ambitious decarbonization targets using hydrogen. All sustainable hydrogen production methods need to be explored and grow strongly to provide the growing hydrogen demand stemming largely from emerging uses in transport, industry or power generation. But green hydrogen is where Siemens is offering the core process technology to produce the hydrogen. This is hydrogen that is produced by renewable electricity powering an electrolyzer to create hydrogen and oxygen from water. If the water and power come from sustainable sources, then the hydrogen is classified as pure green hydrogen, which is the focus of Siemens’ New Energy Business. It sees the market for hydrogen migrating from grey, to blue, and then to green, with each having its own role utilizing different transition technologies.


One key to enabling the potential of the hydrogen economy is a supply chain that can produce green hydrogen at scale and at a price that the market will pay. For the past 10 years that has been a focus at Siemens, and the company is in the formative stages of delivering that technology for the large-scale industrialization of hydrogen.
A big milestone came five years ago with the launch of Siemens’ 1-MW Silyzer 200, (Figure 1) into the market. Since then, the company has undertaken commercial pilots with customers for different applications. Two years ago, it increased the portfolio with a 10-MW version. Last year, together with partners Verbund and Voestalpine, Siemens built its first installation at a steel plant in Linz, Austria.
As with all emerging technologies, the initial cost base was high, with prototypes and bespoke manufacturing, but as the technology matures and adoption increases the costs continue to reduce. As the production volumes grow, advanced automation can be introduced into the manufacturing process along with leveraging digitalization such as digital twins and modularization, where the company uses standardized modular building blocks that are developed to serve for customer use cases.
Much like the famous Moore’s law for integrated electronic circuits, the scale of Siemens’ technology is rising year on year, with an increase in power ratings by a factor of 10 every four years. The company is now in the bidding phase for 100-MW projects, and moving forward it is talking to partners about installations that would break the 1 GW barrier. As time moves on, hydrogen can become as big as wind and solar, but in terms of maturity (market and technology), it is 15 to 20 years behind the more established renewable technologies. Similar cost reductions as have been witnessed in the photovoltaic sector are expected over time.

1. The heart of Siemens’ proton exchange membrane electrolysis plant is the Silyzer module. The innovative technology is ideally suited to exploiting the intermittent generation of wind and solar power. Courtesy: Siemens Energy
1. The heart of Siemens’ proton exchange membrane electrolysis plant is the Silyzer module. The innovative technology is ideally suited to exploiting the intermittent generation of wind and solar power. Courtesy: Siemens Energy


Ultimately, despite the environmental pressures that every facet of industry is under, it will come down to cost. For it to become mainstream, the hydrogen economy cannot be based on subsidies; sustainable markets must be created and getting the cost right is one of the first boxes that must be checked.
The present price of industrial-scale grey hydrogen production is about €1-2 per kilogram (kg), sometimes less, depending on local conditions. When it comes to using hydrogen as a transportation fuel, consumers pay about €9 per kg at a filling station—when they can find one—and that needs to be reduced by at least a third to make it attractive.
When it comes to comparative cost, it makes no sense to compare green hydrogen to conventional fossil fuel options that contribute heavily to global greenhouse gas (GHG) emissions. The mobility sector is on a path to decarbonize and reduce its GHG emissions, so clearly fossil fuels must play a diminishing role.
Today, national climate policy focuses on emissions from light vehicles. In most of the Group of 20 (G-20) member economies, fuel economy or efficiency standards are used to regulate emissions from conventional light vehicles, and 18 of the 20 countries have proposed conventional vehicle bans and/or developed incentives and targets to accelerate sales of lower-carbon vehicles. That is why when considering the fuel needs for light-duty transport, cost and performance of hydrogen-fueled vehicles should be compared with battery-based electric vehicles (EVs).
There are far more EV models available today, and for some consumers, it is a great plus to be able to recharge their cars at home. However, cars powered by green hydrogen are superior when looking at both recharging times and driving range. For example, less than 1 kg of hydrogen is needed to drive 100 kilometers with a medium-sized car, and fueling takes only three to five minutes. This speed is especially attractive for emergency vehicles or taxis that cannot afford to waste too much time charging.
Even more important is the medium- and heavy-duty transport sector, where hydrogen is the most promising zero-emission fuel. Hydrogen’s low weight, long driving range, and fast recharging is especially relevant for heavy-duty vehicles and trains.
However, rather than cost per liter, a more relevant calculation is total cost of ownership (TCO). In its report “Path to hydrogen competitiveness: A cost perspective,” the Hydrogen Council expects that the TCO per vehicle will fall by about 45% compared to current costs at a manufacturing scale of about 600,000 vehicles per year.
For green hydrogen to meet these price points there are three primary challenges that must be overcome: the cost of electricity, the loading factor of the electrolyzer plant, and the capital and operational costs. Moreover,high renewable power generation and transmission capacities have to be built up to provide the electrical power required to produce large quantities of green hydrogen. These are dependent on a variety of factors, some outside the control of the producer, such as the cost of electricity, but with renewable energy becoming a larger part of the energy mix, that factor should take care of itself.
When it comes to capital cost, as with most process manufacturing scenarios, it depends on scale and commercialization of the electrolyzer plant to reduce cost of purchase. As to the operational cost, electrolyzer plant digital twins can be used to optimize the design and to improve the productivity, while maximizing the plant’s lifecycle. In areas that have advantageous conditions, costs to produce green hydrogen could already be about €3 per kg.
Depending on the application, hydrogen can be purified and compressed to levels needed for direct use, storage, or distribution. If storage and transportation is required, there are several options. It can be stored in tanks as a compressed gas or as a liquid, stored in caverns pumped into dedicated hydrogen or in the natural gas pipelines for different applications and if the grid fulfills all technological requirements.
When it comes to transportation the two main methods for transporting hydrogen are tankers for road transportation and via gas pipelines for medium distances, depending on the specific customer use case. When looking at large-scale applications in the hundreds of MW or even GW-scale, it makes the most sense to locate the production near the renewable generation facilities, such as on- and offshore wind parks at locations with very favorable wind resources. This is because the cost of electricity is the major input factor for green hydrogen, comprising more than 70% of the production cost. Because hydrogen is costly to transport, these locations typically require a further synthesis process to green methanol or ammonia, which can easily be transported—these are goods traded globally. This can be perceived as a green hydrogen export business.

The power sector is often seen as a prime target for using green hydrogen to power turbines, but as the share in global CO2 emissions of the power sector is less than 40%, it is vital that it penetrates other sectors as well. Today, there is probably no economically viable business case for producing hydrogen specifically for having it re-electrified directly afterward in a hydrogen-capable gas turbine—and efficiency wise, today it would not make sense either, because there are more applications with higher CO2 reduction potential at lower total cost. But for pilot applications or in a vastly decarbonized world, to further decarbonize the power sector, beyond what can be achieved by installing more renewables, green hydrogen can realize long-term, seasonal power-to-power storage on a large scale.
Re-electrification will be achieved in hydrogen-capable gas turbines, engines, or fuel cells to provide security of electricity supply in periods of low renewable energy supply, such as lack of wind. This use case will become increasingly attractive in the future. It is not an either-or situation, hydrogen (in cars and trucks) can help to decarbonize the mobility sector, decarbonize the industry sector (for example in steel production), and the power sector later.
More than half of global emissions come from industry, transport, or the built environment, so solutions need to be offered to decarbonize those sectors. Traditional renewable energy sources such as wind, solar, and hydro will play their part, but there is also a significant role for hydrogen produced from renewable energy and water, whether used directly or combined with chemicals to create green methanol and green ammonia. These chemicals can be stored, transported, and used in all kinds of sectors as synthetic fuel or fertilizer.
There is keen attention on the transportation sector and there are signs that it could be among the early adopters, especially when it comes to buses, trucks, and trains. Hydrogen fuel cells are already being used for regional trains, which will replace diesel-powered engines. In China, South Korea, and Japan, there is promising growth in cars with hydrogen fuel cells and electric drive trains in cars. In this region, it has been helped by research funding that has increasingly focused on fuel cells instead of batteries. Also, German original equipment manufacturers (OEMs), which are intensively focused on batteries, are developing cars and trucks with fuel cells.
There was a time over the past decade when several automotive OEMs were looking into both fuel cell and EVs, some of them prioritizing fuel cells. The cost of developing two revolutionary concepts in parallel may have driven some to focus mainly on EVs, but now OEMs are returning to the idea of fuel cells. It seems that they are beginning to understand the challenges and limitations of batteries in vehicles.
New development is also being seen in China, where in recent years EVs were supported massively, bringing high growth to the industry. The “Ten Cities, Thousand Vehicles” program that propelled EVs in China is being replicated now for hydrogen transport in cities such as Beijing, Shanghai, and Chengdu.
Going forward, green hydrogen will command a premium price when compared to its less environmentally friendly hydrogen counterparts—blue and grey. The early stages of any technology curve must have some support, much as was seen in the early days of wind and solar power. But in the medium- to long-run, hydrogen must and will stand on its own legs and be viable without external support. When exactly that will happen, depends on several factors, including the adoption rate, economies of scale, and the regulatory frameworks.

“Power-to-X” Decarbonizing Energy with Green Hydrogen: Technology Available and Proven in Production Today

Decarbonizing the world’s economy is possible today by coupling its different sectors to maximize the use of renewable energy resources. The key enabler is Proton Exchange Membrane (PEM) electrolytic technology for generating “green hydrogen” from water at an industrial scale. The hydrogen can then be used as a zero-emissions fuel or combined with other elements as a molecular component in core industrial feedstocks.
With a nearly unanimous consensus of the world’s climate scientists, greenhouse gases — carbon dioxide and methane, chief among them — are causing climate changes that threaten Earth’s viability for humans and must be massively reduced as quickly as possible.
The scale of that imperative cannot be met without the fundamental decarbonization of global power generation, industry, buildings, and transportation by shifting from fossil fuels to renewable energy sources. Together, these sectors account for 95 percent of global CO2 emissions, which exceeded 33 billion tons in 2018, according to the International Energy Agency (IEA).1
While the deployment of renewable energy sources (especially wind and solar) are rapidly rising, they are proving insufficient in reducing global CO2 emissions. As the IEA sees it, the use of renewables must grow much faster if the world is to realize its long-term climate goals.
To achieve these goals, the world needs what’s called “sector coupling.” This enables renewable energy to be transferred to other energy-consuming sectors in the global economy, effectively decarbonizing them, too.
Sector coupling is especially critical to the decarbonization of the world. Renewable energy is making an impact on the power sector; however, the power sector only makes up 40 percent of global CO2 emissions. The remaining 60 percent must also be addressed. One way to accelerate the decarbonization of the other industries is through coupling the use excess green electricity from renewable energy sources to convert various compounds into industrial feedstocks, as well as to displace fossil fuels in other heavy industries and sectors, known as sector coupling.


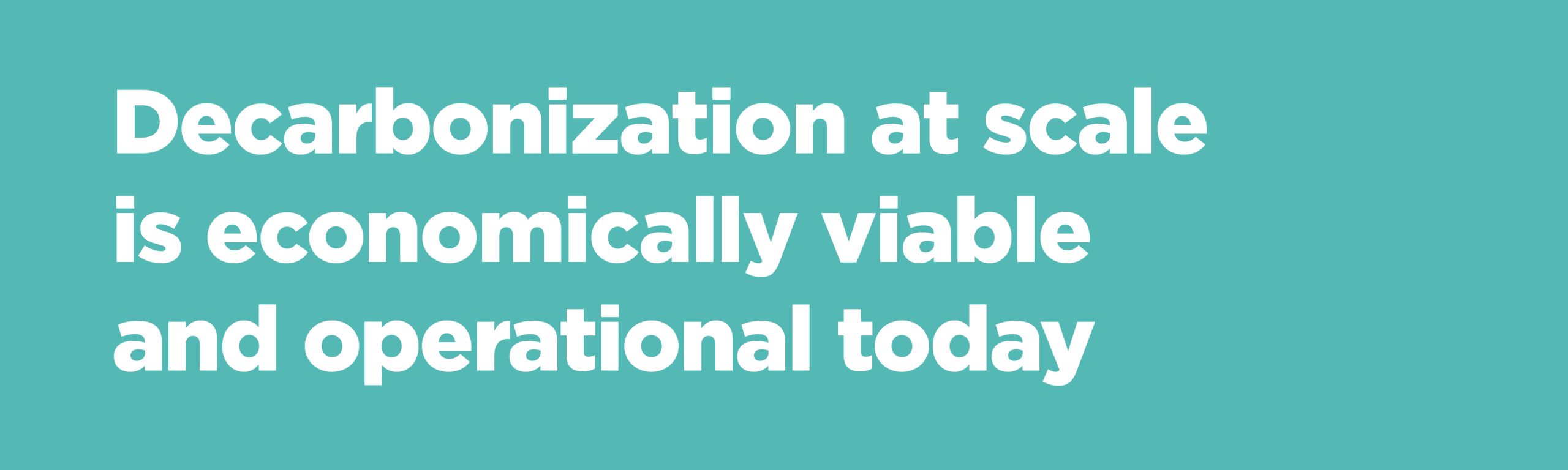
Different energy storage technologies, such as capacitors, flywheels, and batteries, may offer energy portability — especially important for vehicle, marine, and aviation transportation — but they have their limitations in terms of storage duration, scalability, and, in the case of batteries, weight.
Fortunately, there is an alternative energy storage option that shows much promise because of its high energy density, portability, and storability: hydrogen. More specifically, we are referencing “green hydrogen” that is produced using renewable energy sources via advanced electrolysis without carbon emissions.
Hydrogen is the most basic and plentiful element in the universe and powers the sun. By itself or as the basis of synthetic, carbon-based (but neutral) fuels, hydrogen can be integrated into the world’s energy-delivery infrastructure much more easily than other energy storage technologies. Although more work is needed in this area, safety concerns can be addressed with enhanced safeguards added to those used to protect people, property, and the environment from the dangers of flammable fossil fuels today.
To generate green hydrogen at the scale needed to decarbonize the world’s energy, Siemens and key partners have invested for the past decade in generating hydrogen from water, using Proton Exchange Membrane (PEM) electrolysis technology. Simply put, the PEM process uses low-cost renewable energy sources to split water—H2O—into its constituent elements without generating carbon emissions.
Traditional methods of producing hydrogen, such as natural gas reforming (also known as steam-methane reforming) and charcoal gasification, use fossil fuels and therefore generate carbon emissions. In fact, steam methane reforming (SMR) methods using natural gas as feedstock generates 10 kg of CO2 for each kilogram of produced grey hydrogen. This CO2 needs to be captured and stored to make it into emission-free blue hydrogen
In contrast, the PEM process produces green hydrogen, which can be used as an electrolyzed fuel (i.e., “e-fuel”) by itself or, combined with CO2 via methanation, it can produce e-fuels such as methane (CH4) or methanol (CH3OH) for use as an industrial feedstock. Hydrogen can also be combined with nitrogen to form ammonia (NH3) as another important industrial feedstock.
What’s more, green hydrogen produced by PEM electrolysis can be converted into electricity again as an admixture with natural gas or in pure form to fuel gas turbines. In 2019, as part of its commitment toward environmental sustainability, Siemens signed a European industry agreement that promised our new gas turbines can be operated with 20 percent hydrogen (mixed with natural gas) by 2020 and 100 percent hydrogen from 2030 onwards.
Parts of these commitments have already been fulfilled, as much of the Siemens gas turbine portfolio can use fuel mixtures with hydrogen levels of 30 percent or higher, and even up to 100 percent in some turbine models.

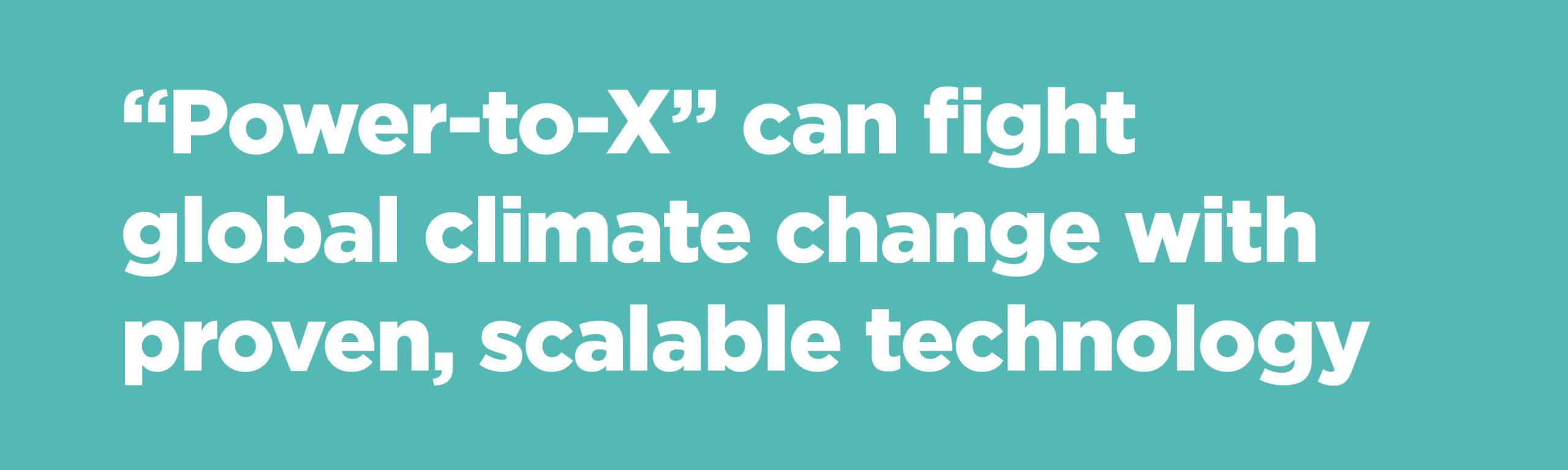
Siemens has long supported a concept related to the PEM electrolysis approach known as “Power-to-X.” It’s often abbreviated as “PtX,” with “X” relating to the fuel-type that’s ultimately produced. So, readers may see variations on this naming convention, such as:
• PtG: Power-to-Gas (gaseous e-fuels, including hydrogen itself or admixed with natural gas)
• PtL: Power-to-Liquids (liquid synthetic e-fuels and industrial feedstocks)
• PtC: Power-to-Chemicals (chemicals for industrial use)
• PtH: Power-to-Heat (via resistance heating or heat pumps)
• PtH2: Power-to-Hydrogen (via PEM electrolysis)
• PtP: Power-to-Power (using PtG or PtL outputs to generate electricity)
In fact, as one of the many activities to decarbonize the world’s energy production and use, Siemens is active in the “Power-to-X for Applications” Working Group at the Mechanical Engineering Industry Association (VDMA), which has 3,200 member companies and is Europe’s largest mechanical engineering organization. Our involvement with VDMA is part of Siemens’ commitment to social and environmental responsibility.
Green fuels can replace fossil fuels, lowering the carbon emissions from their expended energy by as much as 90 percent (or more, in the case of using clean-burning, green hydrogen, with water as the benign by-product of its oxidation). They can also replace biofuels, such as ethanol, which now consumes considerable farmland to grow its main feedstock, corn. This can resolve the food-versus-fuel debates on the use of increasingly valuable farmland and return acreage to food production or non-crop uses, such as nature preserves, recreation areas, and residential developments.

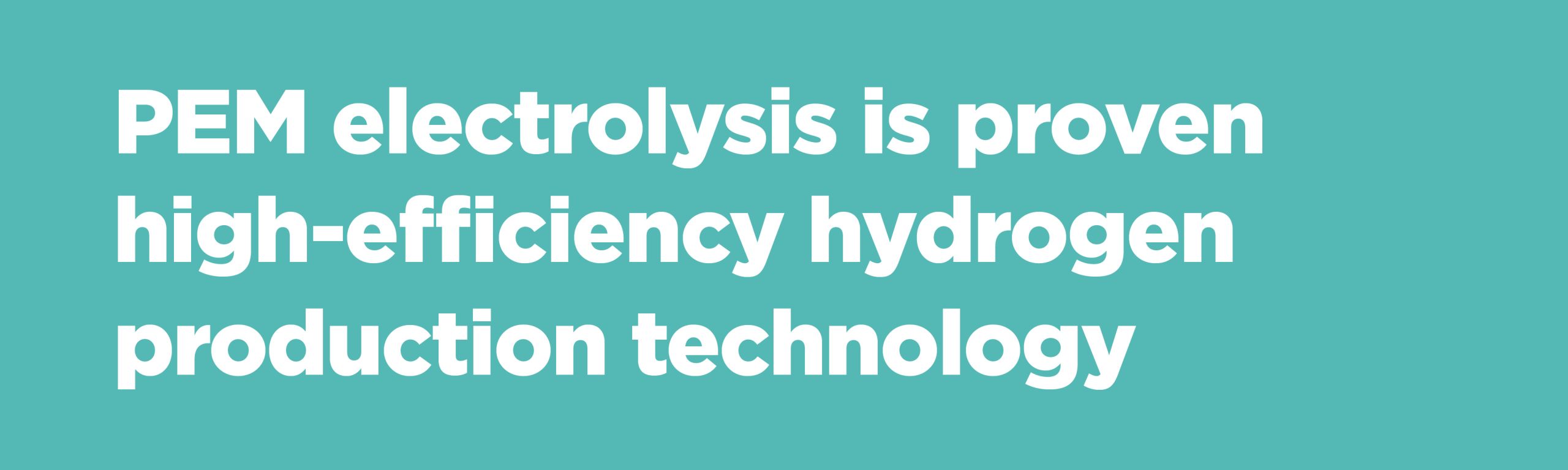
PEM electrolysis uses a cathode-anode cell that features a solid polymer electrolyte that conducts protons, separates water into hydrogen and oxygen, and protects the cell’s electrodes. Developed as a more efficient alternative to traditional alkaline water electrolysis, PEM electrolysis has three main advantages:
• Responsive and flexible. PEM electrolysis can be coupled directly to renewable energy sources. It has black-start capabilities, which means it doesn’t need an external power source to restart from a partial or total shutdown. With an extended operating range, PEM technology can ramp up to 10 percent or more in its operating capacity in less than one second. It can operate from 5 – 100 percent of capacity, providing exceptional operating flexibility.
• Inherently clean in operation. With only water, green hydrogen, and oxygen in a PEM electrolysis system, the technology requires no aggressive chemical electrolytes, such as the potassium hydroxide (KOH) electrolyte required by alkaline electrolysis systems. In addition, it produces hydrogen that is more than 99.9 percent pure — without any CO2 emissions.
• Economically competitive. Compared to the alkaline electrolytic systems, PEM electrolysis has a smaller footprint, and it requires low maintenance, resulting in lower operating expenses and total cost of ownership.
95 percent of today’s global production of hydrogen is done via SMR and coal gasification methods 3 — with both generating CO2 emissions — PEM electrolysis can produce “green” hydrogen at competitive prices when electricity from renewable sources cost less than $0.04/kWh.
In the past, only geothermal and hydroelectric could deliver such low-cost power, but wind and solar costs have fallen rapidly. In fact, a 2019 report comparing different costs of power generation via renewables shows that onshore wind costs of $0.03-0.04/kWh are now possible with the latest generating technology. And, thanks to advancements in photovoltaic efficiencies, new solar PV projects are lowering the costs of electricity to as little as $0.03/kWh. 4
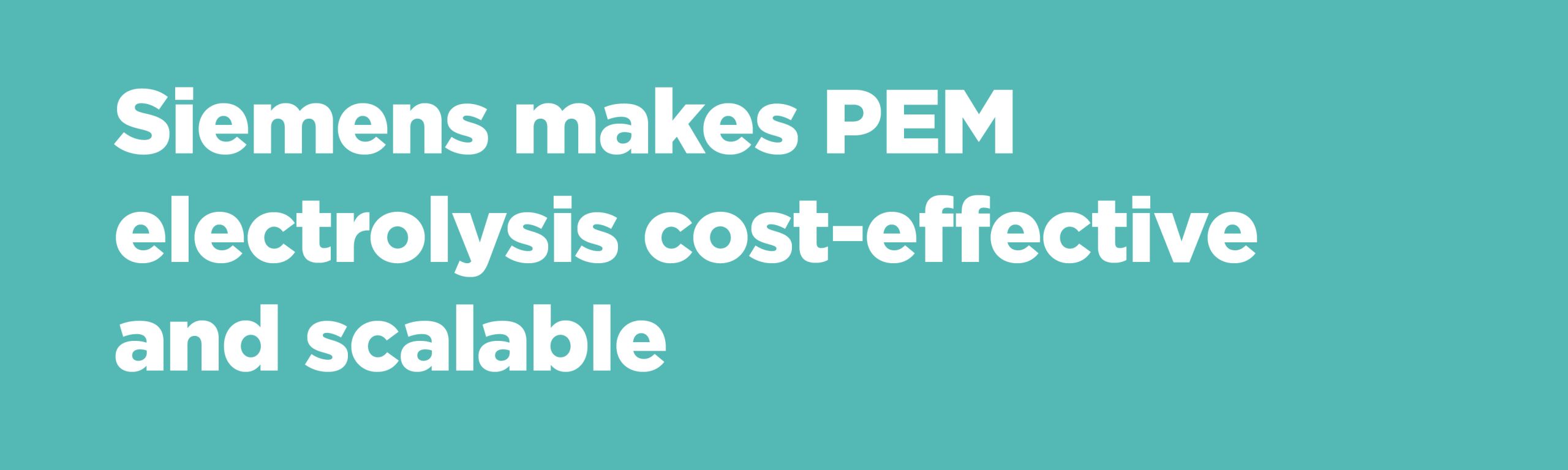
In September 2015, Siemens became the first global industrial company to commit towards carbon neutrality by 2030. To this end, Siemens is one of the world’s leading companies in developing and commercializing PEM electrolysis as a cost-effective and scalable technology to drive PtX initiatives.
Not coincidentally, in 2015 Siemens deployed the Silyzer 200, a large-scale, commercial version of PEM electrolysis that is one of the world’s largest PtG plants in Germany. Today, the company has taken that technology into its third generation with the deployment of the Silyzer 300 at the H2FUTURE project, in partnership with VERBUND Solutions GmbH, voestalpine Stahl GmbH, K1 MET GmbH and the Austrian Power Grid AG.
The Silyzer 300 consists of 24 PEM electrolytic modules that together draw 17.4 MW of power to produce up to 730 pounds (330 kg) an hour of virtually pure hydrogen with no CO2 emissions. The system operates at 75 percent efficiency, which helps cut hydrogen’s production cost by 40 percent and becomes more competitive compared to SMR and coal gasification processes, both heavy CO2 emitters without CCUS application.
The Siemens Silyzer development roadmap targets fourth-generation hydrogen plants that by 2023 can draw more than 100 MW of power for hydrogen production at ever- greater efficiencies. By 2030 and beyond, Siemens envisions building 1,000 MW, fifth-generation plants.
As a leading developer of PEM electrolysis technology, Siemens complements this capability with leadership in the carbon capture, utilization, and storage (CCUS) technology required for many parts of PtG and PtL processes. For example, Siemens can offer the CCUS industry a wide range of electrification, automation, and digitalization products from its vast portfolio, plus global domain expertise and experience, to assist its operators in this regard.
Another key element is the Siemens portfolio of advanced gas turbines, which can effectively recycle the stored hydrogen into electricity by using it as fuel. As mentioned, Siemens is committed to making its gas turbines able to run 100 percent on hydrogen by 2030.
In addition, Siemens Energy is offering a whole range of market leading hydrogen compressors required to move the hydrogen in pipelines or use in chemical processes.
Importantly, Siemens had decades of expertise in the design, engineering, construction, commissioning and lifecycle management of complex power generation and process technology plants. This expertise includes the ability to integrate and bundle most of all the required technologies — especially PEM and CCUS — into solutions tailored for a customer’s specific project need.
In addition, Siemens technologies can help operators drive continuous improvements in system efficiencies and product costs, especially when using volatile solar and wind energy. Finally, Siemens has both the financial resources and global footprint of direct sales and support to be the world’s catalyst for the massive decarbonization needed to successfully fight climate change.
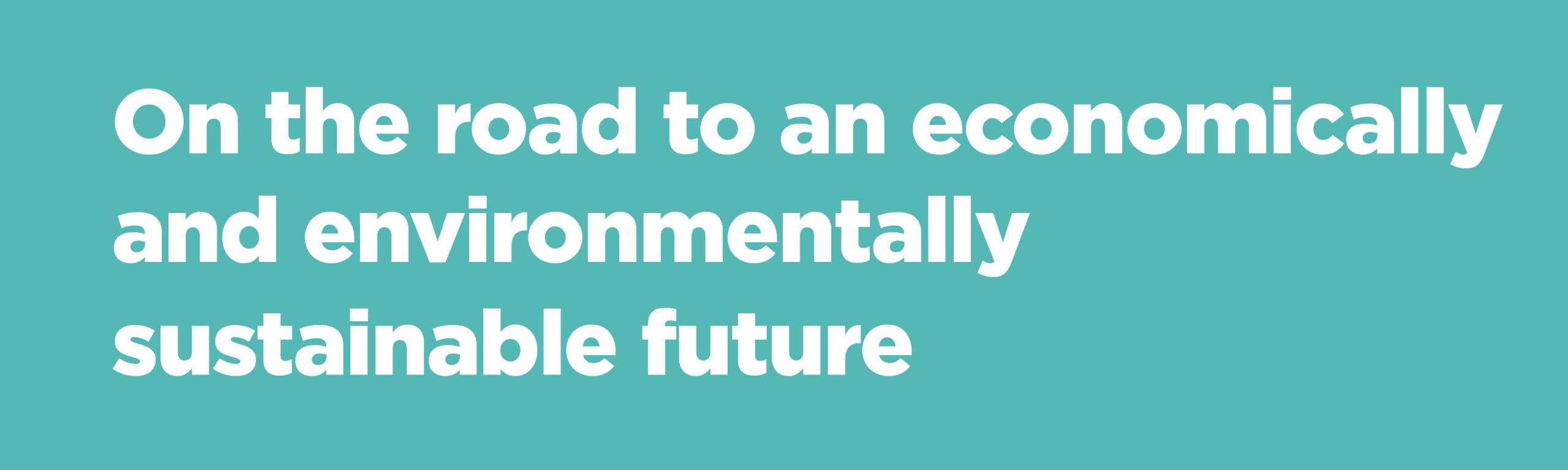
The idea of the “greenhouse effect” goes back to 1824, when French scientist, Joseph Fourier, hypothesized that the atmosphere traps some of the sunlight’s radiant energy much like a greenhouse does. Then in 1896, Swedish chemist, Svante, observed that CO2 was adept at trapping heat radiation and the massive amounts released by burning coal could warm the planet.5
So, while climate change concerns may seem relatively recent, they aren’t. However, more recently there has been an increase in the urgency of warnings from the climate science community that the world must intensify decarbonization of its energy use in the coming decades to prevent irreparable harm to Earth’s climate that could have dire (if not grave) consequences for all living species.
For this reason, Siemens has made strategic priorities out of its involvement in the PtX sector-coupling movement and further development of its Silyzer PEM electrolytic technology for hydrogen production at ever-greater scale.
Although Siemens has considerable technical expertise to help drive both technological and industrial initiatives, the effort to decarbonize the world’s energy use will require the commitment and assistance of both the public and private sectors.
The former needs to develop relevant regulatory and tax incentive frameworks to spur greater participation by the latter. Siemens welcomes both to join in the global effort needed to curtail further heating of the planet and contain the consequences for humanity that are already appearing in the form of extreme weather events and mass migrations of people.
The good news is that the world has a consensus on the causes of climate change, and Siemens has solutions to put it on the road to an economically and environmentally sustainable future. Now we need the widespread support of the private sector to reach our needed destination: a fully decarbonized energy model worldwide.
1 “Global Energy & CO2 Status Report.” The International Energy Agency. https://www.iea.org/geco/
2 IBID
3 Louis Brasington. “The Role of Green Hydrogen in Global Decarbonization.” Cleantech Group. December 13, 2018. https://www.cleantech.com/the-role-of-green-hydrogen-in-global-decarbonization/
4 Dominic Dudley. “Renewable Energy Costs Take Another Tumble, Making Fossil Fuels Look More Expensive Than Ever.” Forbes. May 29, 2019. https://www.forbes.com/sites/dominicdudley/2019/05/29/renewable-energy-costs-tumble/#1dec040be8ce
5 “Harbingers of doom: a brief history of climate change warnings. ” BBC History Magazine. 2005.
https://www.historyextra.com/period/modern/climate-change-warnings-history/