Rediscovering Fire: Advancements in Gas Turbine Combustion
Gas power market drivers and a quest for relevance in future decarbonized systems are driving fundamental shifts in gas turbine combustion design approaches.
Combustion lies at the heart of gas turbine technology. It provides the core function of adding heat, through a controlled burn of large amounts of fuel and air, to a gas turbine’s conversion of chemical energy into mechanical energy and, for power generating turbines, electrical energy. In concept, a combustor essentially comprises a fuel injector and a wall to contain the flame. But far from simplistic, the process must achieve a delicate balance of equivalence ratio between the fuel and available oxygen; flame stability; and operational stability to support acceleration and operation of the gas turbine over the entire load of the machine.
Developers have attempted to refine this complex dance for decades, as John Gülen, a fellow at Bechtel Infrastructure & Power Corp. and the American Society of Mechanical Engineers, and the author of several books profiling the evolution and status of gas turbine technology, told POWER. “It can be traced to Hans von Ohain and Sir Frank Whittle developing their jet engines in their laboratories—pretty much unaware of each other—in Germany and England, respectively, in the 1930s,” he said. Von Ohain, who used hydrogen as fuel owing to its high flame velocity and wide combustion range, achieved good performance at off-design, as well as transient operation (startup and shutdown), and was able to accelerate his engine development to the first aircraft application. “Sir Frank, on the other hand, chose to use liquid fuel and ran into difficulties,” Gülen said.
Even at that time, different designs used cylindrical cans and annular combustion chambers, noted Gülen, who lays out the engineering history in a recent book, Gas Turbines for Electric Power Generation. “The combustors of that era (in terms of architecture, silo-type combustors used in land-based industrial units can be added to the mix) can be characterized by their diffusion type flame structure,” he explained. “Their claim to fame (so to speak) was their stability due to the near-stoichiometric reaction in the flame front (ϕ ~ 1) over a broad range of operability.”
However, their “Achilles’ heel was high flame temperature and commensurately high nitrogen oxide (NOx) emissions,” which technology developers sought to control by injection of a diluent, such as water or steam (in power plants) or nitrogen (in syngas-fired units in refineries). “These combustors could easily burn any fuel gas with compositions including hydrogen as high as 60% (by volume) or even higher,” said Gülen. “Nevertheless, the benefit came at a price—reduced performance [stemming from] steam robbed from the bottoming cycle at the expense of steam turbine output, and water consumption.”
Combustors: The Heart of a Gas Turbine
A combustor—also known as the combustion chamber or “burner”—serves the crucial function in a gas turbine to take in high-pressure and high-temperature air fed into it by the compression system and combust that air with injected fuel to significantly raise its temperature. The combustor then feeds that searing, high-pressure gas stream into the turbine components, where an intricate array of blades spin a generator to produce power (as well as drive the compressor to draw more pressurized air into the combustor).
To achieve the complex balance between a large heat release, fuel variability, compressor airflow and other physical and operational considerations, gas turbine combustor configurations typically fall into three categories though designs differ uniquely by manufacturer.
1. Silo, which is a large single- or double-cylindrical chamber.
2. Annular, or ring combustor, where an annular flame tube is placed within the cylindrical liner or casing. The configuration is found widely in aero-engines as well as aeroderivatives.
3. Can-annular, which features multiple “cans” on a single gas turbine, are the basis of most American designs, but it has also been adopted by Mitsubishi Power, Siemens 60-Hz units, H-class, and Ansaldo’s GT36.
From a flame perspective, combustion systems fall into two categories. Diffusion-type flame, where fuel and air mixing takes place via diffusion, requires a diluent into the reaction zone to control nitrogen oxides. Silo-type combustors are diffusion combustors, featuring a large volume with burners located at the top. But as John Gülen explains in his 2019 gas turbine primer, the increasing turbine inlet temperatures of modern high-efficiency gas turbines and more stringent emissions requirements have “rendered silo-type combustors less and less suitable to the achievement of uniform temperature profiles.”
Premixed flame, which enables dry-low NOx (DLN) technology, involves mixing fuel with air upstream of the reaction zone in fuel-lean conditions to prevent high flame temperatures that create NOx. Gülen said there are three types of premix combustion: lean-lean; rich-lean staged; and lean premixed combustion. “Staging refers to the introduction of fuel into the combustor in the axial direction,” he wrote.
The DLN Evolution
In the 1990s, General Electric (GE) pioneered a breakthrough solution that sought to change the flame structure from diffusion—whereby the fuel is injected unmixed and burns at maximum flame temperature using only a portion of the available air—to a “premix” flame via a process that premixes the fuel and air before they enter the combustor to drastically reduce NOx emissions, as well as using air as a diluent to reduce combustion flame temperatures. Several original equipment manufacturers (OEMs) soon adopted this “lean premixed” combustion process, referring to it through a variety of trade names. GE and Siemens-Westinghouse called it Dry Low NOx (DLN); Rolls-Royce promoted it as a Dry Low Emissions (DLE) process; and Solar Turbines, as a SoLo NOx process.
“In the ensuing three decades, DLN combustors went through several generations (for example, from GE’s DLN 1 to DLN 2.6+) addressing mostly stable operation problems,” said Gülen. However, “the task was made more difficult with increasing turbine inlet temperatures [TIT] in the case of first 60% (net LHV) and nowadays 65% combined cycle efficiency with natural gas firing,” he said. “In comparison to the early days of the DLN technology with TIT of 1,350C–1,400C (vintage F class), we are now looking at ‘advanced class’ gas turbines from the major OEMs (H-, HA-, HL-, or J-class depending on the OEM) with TIT pushing the 1,700C mark.”
At GE, research efforts yielded the DLN-1 solution for E-class turbines, and the DLN-2 solution for F-class turbines; the latter has also been applied to EC- and H-class machines. In 2015, GE introduced a DLN2.6+ combustion system for new and existing 7F gas turbines, and in 2018, it announced a “flex” upgrade solution, which combines the DLN 2.6+ combustor with axial fuel staging (AFS) technology. “This is in contrast to the earlier method of parallel or radial fuel staging, the hallmark of lean premixed combustion,” explained Gülen. “It is interesting to note that axial fuel staging in a single combustor can is an inherent characteristic of gas turbines with sequential (reheat) combustion, e.g., former ABB/Alstom (now divided between GE and Ansaldo) GT24/26 gas turbines.”
More recently, GE has rolled out its DLN 2.6e combustion system—which the 9HA features, but which its newest model, the 7HA.03, will introduce for the first time on a 60-Hz machine when it comes online next year. As Dana Jackson, lead mechanical component owner on GE’s 9HA 2.6e DLN Combustion team told POWER in April, the DLN 2.6e is, at its core, a “redesign of GE’s fuel nozzles”—components that deliver fuel and air in the reaction zone—derived from work with the Department of Energy on its high-volume hydrogen gas turbine program (Figure 1).
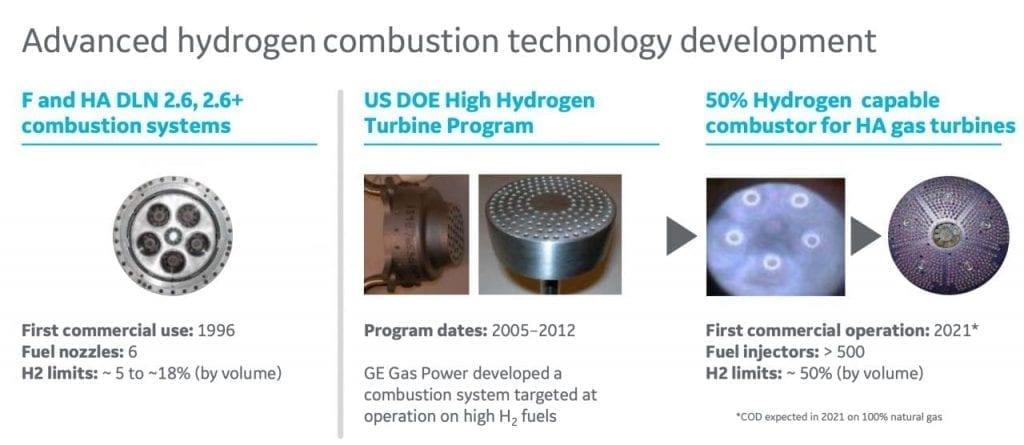
1. GE’s development of a new combustion system capable of operating on high-hydrogen fuels has been ongoing for more than 15 years. Courtesy: GE
John Intile, vice president of engineering for GE Gas Power, also noted the technology gives GE a distinction compared to its competitors because it orchestrates multiple systems. “The big challenge we face with combustion is that it’s like lighting a barbecue in the middle of a hurricane,” he said. “As we continually push firing temperature up at higher pressures and temperatures, it’s getting harder and harder to keep that barbecue lit.”
The DLN 2.6e combustor features an additively printed “micromixer” that “unlocks new ways in which we can mix fuel and air together to be able to drive to higher fire temperatures without the penalty of emissions,” he explained. Paired with an AFS system, which allows an “introduction of almost 40% of fuel into the second stage,” the technology has enabled GE to “really go after the time and temperature aspects of how NOx is created,” he said.
Catering to a Changing Market
Combustion system research and development have been emphatic on flame stability and NOx control, owing largely to the gas power industry’s new transformative role in modern power systems to provide flexibility as a support to increasing shares of renewables, Vinayaka Nakul Prasad, manager of corporate strategy and global lead for Siemens Energy, told POWER in April.
“Gas turbines are increasingly intermittent—they’re backing renewables, they’re having very aggressive startup times, very high cycling of gas turbines, high ramp rates, and even turndowns, which are getting very low,” he said. In addition, customers, who want assurance machines will remain relevant and reliable in decarbonized systems, are calling for wide fuel flexibility, including for low-carbon fuels like hydrogen, he said.
Siemens’ “state-of-the-art” combustion technology is lodged in its newest machine, SGT6-9000HL (Figure 2), a 60-Hz version which last year began a four-year real-condition test program at Duke Energy’s Lincoln Combustion Turbine Station near Denver, North Carolina. In June 2020, Siemens also sent a 50-Hz HL gas turbine to SSE’s Keadby power plant in Lincolnshire, UK, for testing and validation before it goes online in 2022. Efficiency gains reaped in that machine came from ramping up firing temperatures from 1,500C to well above 1,600C. “That required aero design changes, including fuel-air mixing and cooling air optimization to keep the primary zone flame temperature low and avoid local hotspots to keep NOx low; looking at heat distribution, making sure parts are really fulfilling their lifetime requirements, considering [the need for] these new ramp rates,” said Prasad.
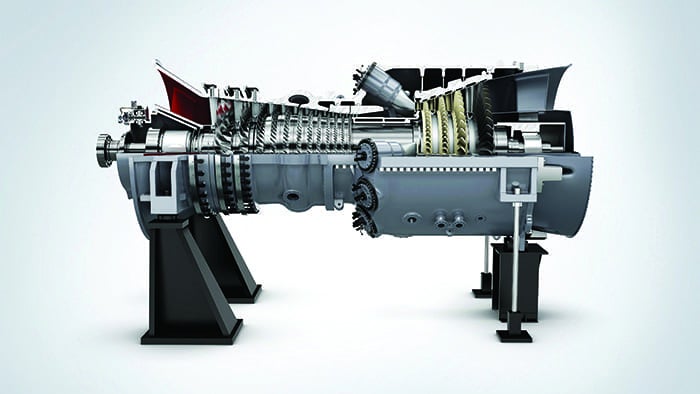
However, Siemens continues to refine its combustion technology, mainly to enable 100% hydrogen combustion by 2030 as part of a commitment set by EUTurbines—a European industry association that includes several other gas turbine manufacturing giants, including Ansaldo Energia, MAN Energy Solutions, and Mitsubishi Power. So far, the company has leveraged crucial experience it gained over decades in unabated diffusion flame, wet low emissions, and dry low emissions (DLE) combustion technologies. “Our heavy-duty fleet can already burn 30% on DLE today. Selected industrial GTs like the SGT-600 can burn up to 60% on DLE,” he said.
A key hurdle is that flame temperatures for hydrogen under adiabatic and stoichiometric conditions are almost 300C (572F) higher than for methane, Prasad explained. Compounding this is that while hydrogen’s laminar flame speed is more than three times that of methane, the autoignition delay time of hydrogen is more than three times lower than methane. Siemens is now working to achieve a fine balance between controlling the flame of the highly reactive fuel and maintaining the integrity of the combustion system—all while attempting to reach the desired level of emissions, Prasad said.
“We’ve got a good grasp on that [owing to] commercial design tools [like] high-fidelity and time-resolved CFD [computational fluid dynamics] simulations and additive manufacturing now capable of printing the required innovative designs. That gives us a headstart when we go into the testing phase at engine conditions, which is an essential step in verifying and further optimizing these designs” he said.
Combusting Decarbonized Fuels
Italian gas turbine manufacturer Ansaldo Energia, too, told POWER its ultimate target for its combustion technology is to enable efficient, flexible, ultra-low emission gas turbines, which will be key to meet global challenges of reliable energy production and to minimize the environmental impact of the power sector. “We see a strong trend towards extending the fuel flexibility of gas turbines to incorporate green fuels, particularly hydrogen and ammonia,” it said.
Ansaldo Energia’s AE94.3A engine has already acquired broad experience in the field, “running for several years with various blends of hydrogen and natural gas,” it said. Recent high-pressure tests have also shown a possibility to operate at increased hydrogen content with minimal or no penalties on emissions, the company said.
Ansaldo noted that it has achieved technology boosts mainly through increased firing temperatures, which in turn “requires short combustion chambers and rapid mixing, so that the post-flame residence times are sufficiently low to minimize detrimental NOx emissions.” In a traditional premix gas turbine combustor, a change in fuel reactivity implies a change in flame location, the company explained. In particular, higher fuel reactivity forces the flame to move upstream, increasing NOx emissions, and potentially overheating the burner. Lower fuel reactivity has an opposite effect, pushing the flame downstream, but it increases carbon monoxide and unburned hydrocarbons emissions due to the insufficient burnout time.
Sequential combustion overcomes this issue by adapting the flame temperature of the first stage (FS) while keeping the overall combustor exit temperature constant, the company said. In a sequential combustion system (as applied in Ansaldo’s GT26 and GT36 engines [Figure 3]), the first-stage premix burner utilizes aerodynamic structures to stabilize a propagating flame. That provides “excellent flame stability and combustion efficiency over an extensive operational range,” the company said. The second stage, in contrast, is primarily auto-ignition controlled. “These two complementary methods of controlling the flames provide a substantial advantage in fuel flexibility. Particularly, it enables DLN combustion of unprecedented amounts of hydrogen in the fuel; such systems are envisaged to be optimized to reach 100% hydrogen combustion for both new units and retrofits.”
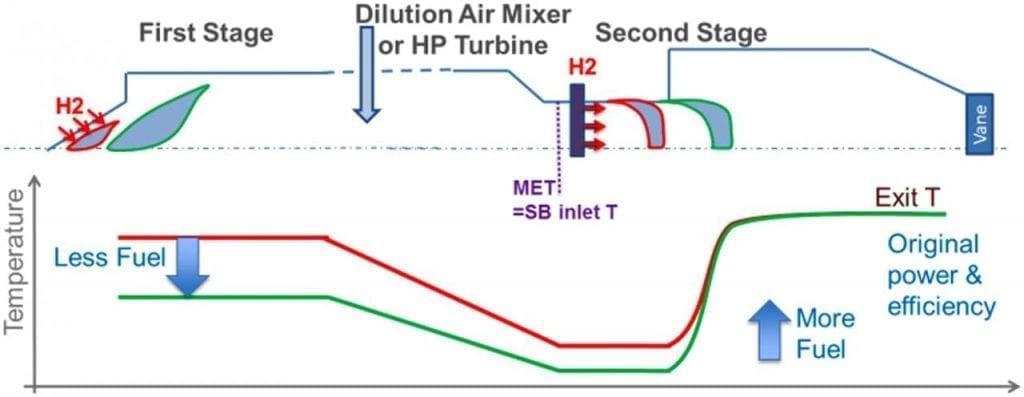
3. Ansaldo Energia’s GT26 and GT36 gas turbines feature sequential combustion technology, enabling higher firing temperatures, lower emissions, and increased operational and fuel flexibility. In the GT26, sequential combustion is implemented with two combustion stages separated by a high-pressure turbine: the first stage (EV) operates at pressures over 30 bar, while the second stage (SEV) operates at roughly half of the EV pressure. In the GT36, no high pressure (HP) turbine is implemented, but the sequential combustion concept is maintained. Courtesy: Ansaldo Energia
In a sequential combustion engine, notably, the second combustor can also be switched off, “allowing the engine to be parked at very low load without penalties on emissions. This is an unbeatable advantage during the times when renewables satisfy the energy demand. In this scenario, the gas turbine can be operated at very low operational costs. When required it can respond fast and deliver power promptly, without any need for a startup procedure,” it said.
Likewise, Mitsubishi Power’s M501JAC gas turbine model integrates a pre-mix combustor technology to address flashback (backfire), combustion pressure fluctuation, and NOx emissions, and the J-series model is already capable of combusting a mix of natural gas and up to 30% hydrogen, it said. To get to 100% hydrogen capability, technology developers are now exploring a “multi-cluster” combustor technology that enables efficient mixing of hydrogen and air via an upgraded fuel delivery nozzle design.
Mitsubishi Power Americas CEO Paul Browning in February told POWER the company is targeting development of 100% hydrogen combustion technology by 2025. “Our view is that natural gas is going to be a transition fuel, but it’s very important to us that our customers know that any gas turbine they purchase from us is not only capable of using 30% hydrogen today, but it’s going to be capable of 100% hydrogen in the future,” Browning said.
However, in tandem, Mitsubishi Power is also developing a 40-MW ammonia-capable gas turbine, targeting its commercialization around 2025. Because ammonia has a low combustion speed, it requires a much larger combustor, and because ammonia contains nitrogen, any system using it as a fuel will need to tackle the “fuel NOx” it generates, it said. The company has explored lowering the NOx via two-stage combustion, but it said larger gas turbines posed “many technical problems, such as upsizing and complication of the combustor.”
The Quest for Industrial Gas Turbine Flexibility
From an industrial gas turbine perspective, MAN Energy Solutions told POWER its combustion technology priorities extend to fuel flexibility, specifically for hydrogen and higher hydrocarbons. “The latter are often present in fracking gases and shale gases. Within the same project, our company is also working on the development of a low-emission premixed combustor, which will be able to burn 100% hydrogen with ultra-low NOx emissions,” it said.
MAN’s flagship technology is the Advanced Can Combustion (ACC) system (Figure 4), which applies advanced lean premixing to reduce the peak flame temperature in the main reaction zone. “The basic aim is to achieve a maximized fuel flexibility with extremely low emissions at wide load and ambient temperature ranges,” it said. The company noted it recently introduced dual-fuel capability for its MGT gas turbine lines—which can already combust a hydrogen volume of 20% in natural gas—to allow for the use of liquid fuel as backup fuel. “This capability increases the reliability of power production in case of shortages of natural gas,” it said.
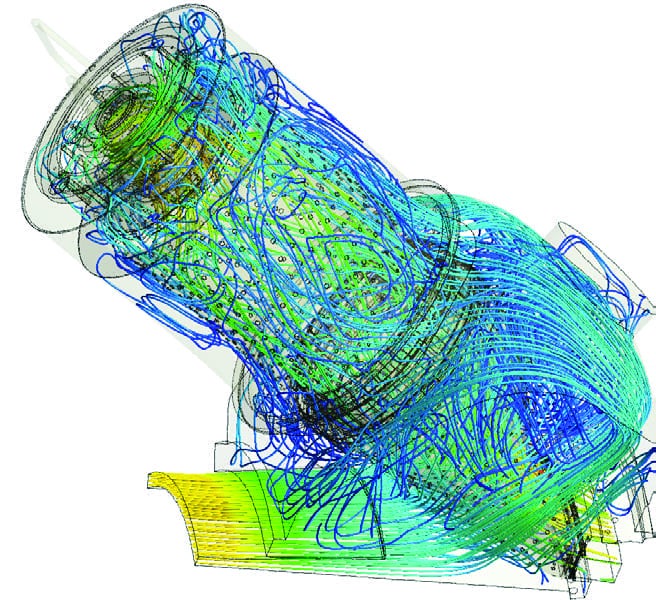
Smaller OEMs, too, are making crucial headway on advancing combustion technology. A notable example stems from a collaboration between Japanese technology conglomerate Kawasaki Heavy Industries (KHI) and Aachen, Germany–based engineering B&B-AGEMA to refine a micromix combustion technology developed by Aachen University of Applied Sciences in the 1980s. As B&B-AGEMA’s Jens Dickhoff told POWER on May 1, the patented technology relies on “multiple miniaturized diffusion flames, which simultaneously prevents flashbacks and suppresses NOx production.”
In July 2020, KHI, backed by the Japanese New Energy and Industrial Technology Development Organization (NEDO) announced the successful demonstration of a DLE, 100% hydrogen-fueled gas turbine under commercial operating conditions in the port of Kobe, Japan. Verification on the gas turbine combustor, part of an innovative 1.1-MWe (2.8-MWth) cogeneration system developed by Obayashi Corp. that integrates a heat recovery steam generator, was slated to end in February 2021.
The Next Frontier of Combustion Advancements
According to a 14-member all-engineer committee that authored a pivotal publication identifying gas turbine technology improvements that can be achieved by 2030 for the National Academies of Sciences, Engineering, and Medicine last year, the future of combustion is rooted in a better understanding of fundamental combustion properties.
While more stringent emissions requirements pushed OEMs to understand flame stability, emissions, turbulent combustion, and combustion chemistry, more recently, “greater interest in improving the efficiency of gas turbine technologies has pushed research and development to understand these issues at increasingly severe pressures and temperatures.” In addition, interest is growing “in cycles that approximate constant volume combustion, such as pulse-detonation or rotating detonation engines,” the committee said. “This has motivated work in detonation limits, detonation wave dynamics, and the transition from deflagration (in which combustion propagates at subsonic speeds) to detonation (in which combustion propagates at supersonic speeds).”
“The conventional Brayton cycle, which is the basis of the present gas turbine technology, is based on the (ideal) constant pressure heat addition process. In practice, this is accomplished in a combustor with a relatively small pressure loss, e.g., 5–6%,” explained Gülen in April. “There is, however, another ideal cycle, which is superior to the Brayton cycle in thermal efficiency, in which the heat addition is at constant volume. In this process, cycle heat addition (i.e., combustion in the actual hardware) results in temperature and pressure increase. Thus, one can achieve high turbine inlet temperature and pressure without the high parasitic power consumption of the compressor (which eats up nearly 50% of the turbine output in a conventional gas turbine operating in Brayton cycle).”
This next frontier in gas turbine cycle and combustion technology, which Gülen calls “pressure gain combustion,” is currently dependent on detonation combustion, though it is an “imperfect solution to this vexing (and very complex) engineering problem,” he said.
“Earlier, with a big focus on aircraft propulsion, the proposed solution was the pulse(d) detonation combustion (PDC), which was based on generating detonation waves in a multi-tube design creating an intermittent (i.e., pulsating) gas flow (up to 100 Hz in intermittency as an approximation to steady turbine inlet gas flow),” he continued. “Recently, PDC fell from favor and was replaced by another detonation combustion technology, namely, rotating detonation combustion (RDC), which is supposed to overcome the practical problems presented by PDC due to its unique construction and operating principle.”
However, its practical implementation is still in the future. Still, for land-based power generation gas turbines, pressure gain combustion, via PDC or RDC, presents an opportunity “to increase the combined cycle efficiency (realistically) by two percentage points without pushing the TIT to very high levels,” said Gülen. “Furthermore, many of the practical difficulties and risks associated with a continuously exploding contraption in the limited space of an aircraft with all concomitant weight, size, vibration, etc. problems are much easier to overcome in a land-based machine.”
Over the near-term OEMs are focusing efforts on streamlining component performance. At GE, which is pushing for a gas turbine system efficiency “north of 64%,” additive manufacturing, including printing parts via direct metal laser melting (DMLM), has allowed it to “produce some of that combustion architecture that we really couldn’t make before in the past,” said Jackson. “In the last five years, we’ve put a lot more additive made parts into our combustion systems, and we have the most now with our DLN2.6e,” she said.
Meanwhile, Intile suggested GE is “at the beginning of its technology S-curve with its DLN2.6e,” he noted, “The real challenge we face now is in material systems.” Because GE’s emissions capability has essentially “leapfrogged its materials capability, we are now investing in R&D, in new coatings and new materials within the combustion system so we can catch up to the emissions capability,” he said. “So I do believe there’s still an opportunity to go beyond today’s efficiency levels even further.” ■
—Sonal Patel is a POWER senior associate editor.
Editor’s note: This article is an extended version of POWER’s May 2021 print cover story.